Solving the near-term CO2 storage gap
Alternatively titled "How to scrape by while waiting for Class VI wells to come online"
Direct air capture (DAC) is scaling rapidly, and new mid-scale facilities capturing thousands to tens of thousands of tons of CO2 per year coming online over the next few years will start to require financing beyond venture capital. Robust offtake agreements will be required to secure financing, but these offtake agreements require defensible plans for CO2 storage. In the U.S., most CO2 storage must and will ultimately occur in Class VI wells. However, very few Class VI wells are currently active, and integration opportunities may be limited in the short run. This article summarizes different options that DAC startups have to resolve this issue ranging from integrating into multi-modal transport and storage networks to leveraging mineralization opportunities and beyond.
Three Chicken-and-Egg Problems
DAC developers face several chicken-and-egg problems. This kind of problem is common in all new industries but is particularly salient for DAC given the multiple conditions required for it to work and scale. These include (a) gaining access to supply chains that provide custom materials and equipment at low costs; (b) securing dedicated, additional sources of low-carbon energy to power the systems; and (c) finding ample, secure storage for the captured CO2. Each of these inputs are required to help proposed DAC projects have a chance of minting certifiable credits at reasonable prices, allowing the facilities to secure the financing necessary to commence construction. However, all these conditions are difficult to secure without the industry first being at scale, resulting in a chicken-and-egg situation.
In 2050, when DAC is hopefully operating at a global capacity of hundreds of millions to billions of tons per year, these problems will be resolved. Supply chains for components ranging from sorbent chemicals to monoliths to vacuum pumps and beyond will already have been established for the most part and may only require modest growth and adjustments after this point. The electrical grid in much of the world will be approaching 100% clean even accounting for existing and additional DAC-related load growth. Even if this is not the case, the maturity of DAC projects and expected abundance of clean, firm power options ranging from geothermal to nuclear and so forth should easily enable behind-the-meter clean power deployment to power DAC. Billions of tons of annual capacity of geologic CO2 storage should also be online by then for that will enable storage of CO2 captured from point sources, DAC systems, bioenergy with carbon capture and storage (BECCS) projects, and perhaps even direct ocean removal plants.
To get to this point, though, DAC needs to start scaling now, in 2024, when these inputs are much harder if not impossible to access for new, first-of-a-kind projects. Startups have no choice but to trudge through solving their supply chain woes, which involves options ranging from designing systems to use more commodities and off-the-shelf equipment to simply absorbing high costs for specialty, low-volume materials and equipment. I have written before about different approaches that early DAC projects can take with respect to clean energy procurement, and there is increasing movement toward using annually matched renewable energy certificates for now while phasing in stricter requirements for 24/7 carbon-free energy as the industry matures and scales.
This article addresses the final chicken-and-egg issue proposed here, which some in the carbon dioxide removal (CDR) space call the “storage gap” or the “near-term storage gap.” While being written from a U.S. perspective, the issues and opportunities outlined in this article are applicable to most regional contexts. The guidance offered here could also be valuable for other CDR processes including direct ocean removal and small-scale BECCS.
A Short Primer on Class VI
CO2 captured via DAC can be durably stored in several ways that range from as a solid in building materials to as a supercritical fluid underground. While storage in solid carbonates has potential as discussed below, physical and market capacities limit this approach relative to the gigatons of underground storage available. In fact, there is more than enough underground storage capacity for far more CO2 than humanity has ever emitted! Due to this sheer capacity and low expected costs, underground injection of captured CO2 is likely to outpace other uses and disposition pathways.
Underground CO2 injection in the U.S. must be done in a Class VI well permitted by either the Environmental Protection Agency (EPA) or a state with permitting primacy over this well class. Class VI is one of the underground injection control (UIC) well classes defined by the EPA. Full coverage of the background, nuances, and controversies associated with this well class can be found in a series of articles from Jack Andreasen starting here and in various other sources. The EPA maintains a handy tracker showing progress across all projects and wells undergoing the federal permitting process. Trackers can also be found on the websites of states with primacy over Class VI permitting, which currently include Louisiana, Wyoming, and North Dakota.
For this article, it is important to know that (a) there are only a handful of Class VI wells in the country that are operating or close to operating with between 150 and 200 more individual wells in various stages of the permitting process; (b) it takes at least several years if not longer to reach a final permitting decision on a well, during which no CO2 can be injected; and (c) annual expected CO2 storage volumes are often in the hundreds of thousands to millions of tons per year. Much of the storage capacity for a well needs to be contracted or at least highly likely to materialize to secure financing for the expensive development process spanning characterization to permitting to construction and operation.
While many wells will be coming online in the next few years, with many funded by the Department of Energy’s CarbonSAFE program, this may not be a panacea for DAC’s storage dilemma. Smaller projects deployed over the next few years and perhaps even later may need to find alternate solutions to expedite timelines, resolve uncertainties, and gain locational flexibility for siting. Below are eight sometimes-related options that DAC developers can consider to address the near-term storage gap with examples to serve as inspiration.
Develop a Dedicated Well
Some DAC companies may choose to develop a dedicated well, likely in tandem with an experienced storage developer. While this solution would be tidy and the most desirable in terms of maintaining siting flexibility, it is difficult to operationalize in the near term as permitting will take years and Class VI well capacities generally do not align neatly with expected mid-scale DAC scales. The other solutions described here are necessary to consider as this is not often viewed as a reasonable option.
Individual DAC systems generally need to scale up in stages, from lab scale to pilot scale to mid-scale to large scale. Mid-scale DAC facilities capturing thousands to tens of thousands of tons per year need firm revenue sources to secure financing for upfront costs that start to run in the tens of millions of dollars. Securing revenue from CDR credits requires actual storage of the CO2, but a dedicated well likely cannot be developed at the needed scale or on a reasonable timeline for this size of a DAC plant.
Theoretically, a well with a larger capacity than that of the initial DAC facility could be developed for a plant that intends to scale up operations over time. Before being canceled/relocated, CarbonCapture Inc.’s Project Bison was expected to scale up in stages while storing in a Class VI well with Frontier Carbon Solutions. However, without additional CO2 providers in the network as discussed below, the DAC company and project may not be mature or creditworthy enough to convince a storage developer to take this level of risk for a single project.
Larger incumbents operating in the DAC space, such as Occidental Petroleum/1PointFive, might have an easier time navigating the permitting process and securing dedicated wells. EPA recently approved draft permits for three Class VI wells in Texas tied to 1PointFive’s STRATOS project, although notably this project at an expected capacity of 500,000 tons per year will be much larger than any mid-scale DAC facility coming online over the next few years.
On the startup side, Spiritus announced that they filed a Class VI permit in Wyoming for a project that could eventually capture and sequester up to two millions tons per year, but the sequencing of this project and interim developments is uncertain. Will the well(s) be developed in stages to accommodate increasing DAC capacities? Will the DAC system somehow be able to jump from a lab- or pilot-scale system right to a megaton-scale one to match the well capacity? What other CO2 sources might want or need to participate, and what effect will this have on project implementation? These are the kinds of questions that companies attempting this approach will need to answer.
Tap into an Existing or Soon-to-Exist Transport and Storage Network
Rather than developing an exclusive well, DAC projects may be able to tap into existing or developing transportation and storage networks that are primarily being built out for point-source capture. This approach leverages existing work, well applications that have often already moved through much of the permitting process, and significant capacities of more technologically mature point-source capture projects. My personal prediction is that this strategy will be the most popular in the medium term as more Class VI wells and pipeline networks come online and as DAC projects advance to mid-scale capacities.
While potentially convenient, this approach is not without shortcomings. For one reason or another, DAC projects may not be able to be sited close to the new wells, meaning that transportation via truck, rail, barge, ship, or pipeline may be required. Each transportation option adds costs and extra engineering considerations and challenges. For example, many pipeline systems are not necessarily designed to handle intermittent flow, but DAC systems may supply CO2 intermittently depending on the technology and system design. Research has shown that such issues are surmountable, but solving them may add complexity, uncertainty, time, and thus substantial cost to projects.
Additionally, there are social and reputational risks to consider. Pipelines and other means of chemical transportation are controversial and have a mixed record, and an increased reliance on them (relative to captive use, which for DAC would involve onsite capture and storage) poses increased risks to society and to the projects to which they are tied. Closer ties to point-source capture, whether objectively valuable or not, could also pose additional reputational risks given environmentalist resistance to the technology.
For their announced ~320,000-ton-per-year DAC project in Northwest Louisiana, Heirloom intends to leverage this strategy in tandem with storage developer CapturePoint at the Central Louisiana Regional Carbon Storage Hub. Also in Louisiana, Climeworks has announced intentions to work with Gulf Coast Sequestration for Class VI storage to support their Project Cypress work in the area. DAC company Heimdal has also noted intentions to work with CapturePoint on their Class VI developments in the Osage Nation in Oklahoma. As noted, I predict there will be many more such announcements over the next few years and that the issues outlined here will be resolved on way or another.
Aggregate Storage Demand
The above strategy essentially entails a hub model, where multiple industrial facilities are sited close together to share resources and exploit synergies (to learn more about the origins of the hub concept and industrial symbiosis, read up on the Kalundborg Eco-industrial Park). In this case, it would involve transporting CO2 from both point-source capture and DAC to a common storage site.
In addition to this model, multiple DAC systems from different technology providers could be bundled at the same site to ensure enough capacity to align with a larger Class VI well capacity. This may be a necessary step before any of the participating DAC technology providers could deploy at a larger scale on their own. It could also be structured to provide additional benefits above and beyond easier storage access including shared balance-of-plant equipment (e.g., dehydration, oxygen removal, compression) that benefits from economies of scale, exchange of utilities like water or waste heat, shared labor costs, knowledge exchange, and even bundled procurement of energy or materials to secure volume discounts.
Challenges would include negotiating host site ownership, managing intellectual property rights, coordinating scale-up plans, engineering integrated systems, and managing the competitive relationship between participating DAC companies. Deep Sky is taking this approach with their development of Deep Sky Labs, which will bring together up to 10 DAC technologies at the same site and provide bundled access to geologic storage through a partnership with Bison Low Carbon Ventures. The Department of Energy’s Regional Direct Air Capture Hubs program may result in additional examples of this model.
Leverage Enhanced Oil Recovery in Class II Wells
Moving away from Class VI wells, DAC projects can provide CO2 for use in enhanced oil recovery (EOR) in Class II wells, which are wells used to inject fluids for oil and natural gas production. EOR, also known as tertiary recovery, is a process used for decades by upstream oil companies that involves using thermal, chemical, gas injection, and other methods to recover additional oil from oil wells. This process can inject CO2, although most of the CO2 currently used for EOR is derived from natural, lithospheric deposits resulting in little tangible climate benefit over conventional oil production. CO2-EOR is currently used to produce hundreds of thousands of barrels of oil per day in the U.S., demonstrating its status as one of the only technologically mature CO2 utilization pathways.
CO2-EOR’s environmental value proposition is messy and controversial. As it relates to DAC, Wil Burns from American University defends it here, and Pete Psarras from the University of Pennsylvania complicates it here. Many of the arguments in favor of EOR boil down to recognizing that if we are going to produce oil anyway, it is probably better to inject CO2 down with it while maybe also extending the life of existing wells to reduce the need for new ones. EOR can also provide an immediate revenue source that can help get DAC projects off the ground and down the cost curve, allowing more CDR credit buyers to more easily enter the market and wean DAC off of EOR and associated revenue.
However, if CO2-EOR makes oil extraction more efficient and leads to cheaper oil, then we need to consider the emissions impacts from any induced oil consumption on the margin. The risk that use of DAC CO2 for EOR provides a continued social and economic license for oil production also cannot be ignored. At the very least, combining EOR and DAC creates reputational liabilities that could compound negative perceptions from any involved CO2 transportation. There is a reason that DAC projects like Project Cypress explicitly note that captured CO2 will not be used for this purpose.
Due to these issues, many voluntary CDR credit buyers and monitoring, reporting, and verification (MRV) protocol developers are hesitant to engage with DAC credits generated with EOR. While EOR revenues in tandem with 45Q can provide decent income, it may not be enough to justify construction given the high costs of early-stage DAC and the high prices being paid for non-EOR DAC credits.
With this said, carbon-negative oil—which involves compensating for all the life cycle emissions from produced oil with an equivalent amount of DAC and CO2 storage—could emerge as a potential monetization strategy outside of direct CDR credit sales. Occidental Petroleum and ENEOS are attempting to commercialize related products. Some emerging analysis also indicates that in some cases such as aviation, pairing continued petrochemical use with CDR may be an economically and environmentally preferred means of reaching net zero relative to direct decarbonization. If so, then using EOR with DAC CO2 to obtain this last bit of needed oil could ultimately be the optimal outcome at net zero and beyond.
Regardless of how desirable this solution is for addressing the near-term storage gap, it is a solution. While there are only a handful of permitted Class VI wells out there with maybe a couple hundred in the pipeline, there are over 180,000 Class II wells in the U.S. While not all of them are necessarily suitable for CO2 injection, the sheer number indicates increased familiarity with the corresponding permitting and operation processes that could result in significant optionality for DAC projects.
Heimdal has chosen this route in partnership with CapturePoint for Project Bantam, which is their active 5,000-ton-per-year pilot. Occidental Petroleum/1PointFive’s STRATOS project will also use some of its captured CO2 for EOR, although interestingly some of their press releases announcing DAC credit sales from the project explicitly note that EOR will not be tied to the purchases.
Store in Concrete or via Ex Situ Mineralization
Instead of being geologically stored or used for EOR, captured CO2 can also be converted into a solid carbonate for storage. This process often involves reacting CO2 with an oxide or hydroxide, such as calcium or magnesium oxide or hydroxide, to form a stable carbonate. These carbonates can potentially be sold for construction purposes or simply disposed of in what are essentially landfills or old mine sites. This can occur through storage in concrete, which contains cement that is naturally reactive with CO2, in purpose-mined minerals such as olivine, or in industrial alkaline feedstocks including fly ash, steel slag, cement kiln dust, municipal solid waste incineration ash, waste concrete, and mine tailings.
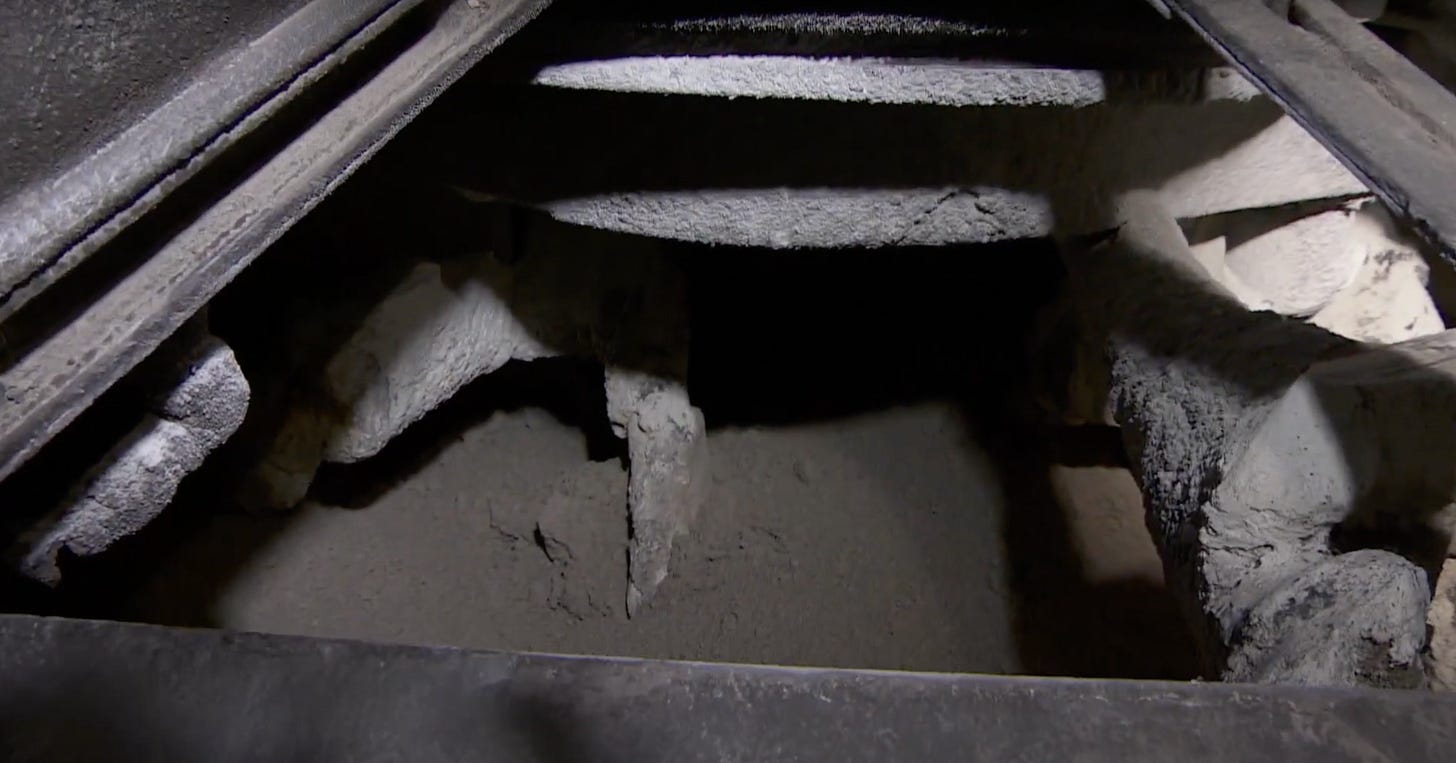
Utilizing this approach could avoid issues with Class VI well permitting and EOR while also potentially providing salable end products like construction aggregates that would generate additional revenue. As explored by Wilcox et al. (2017) and Kelemen et al. (2020), it may also be possible to produce lower-purity streams of CO2 from DAC systems and directly mineralize them, which would reduce capture and potentially final removal costs. DAC startup Clairity Technology has signaled an intention to explore this approach with their system. Other companies such as Blue Skies Minerals and Earth Repair are seeking to directly mineralize alkaline feedstocks with atmospheric air, cutting regeneration of CO2 streams out of the picture entirely!
While promising, this approach faces several hurdles. The first is that corresponding MRV protocols from protocol developers like Isometric and Puro, and any related verification activities, are either still developing or require measurements and calculations that may not be robust in all cases. This is additionally complicated by uncertainty of long-term storage if the CO2 is stored in materials such as construction aggregates that are then sold into the general market. Any uncertainty in terms of MRV protocol compliance threatens the ability to secure offtake agreements and thus financing for the project.
There are also some questions with respect to the additionality of ex situ mineralization. Concrete carbonates naturally throughout its lifetime, and most other alkaline feedstocks would also eventually react with CO2 in the ambient air, meaning that upfront carbonation may only be bringing CO2 storage forward rather than additionally creating it. Further work is required to determine, measure, and describe the true additionality of such processes, which introduces additional risk. System boundaries also play a complicating factor; the industries that produce most alkaline feedstocks generally emit far more than can be stored via CO2 mineralization of their wastes, complicating the overall path to society-wide net zero.
An additional practical barrier to implementation of this approach is capacity constraints depending on the amount of concrete or other feedstocks that are available for mineralization in a given area. DAC plants already need to manage many trade-offs when siting, and planning around feedstock availabilities further complicates both initial siting and scale-up plans.
For their 1,000-ton-per-year facility in Tracy, California, Heirloom is storing captured CO2 in concrete in partnership with CarbonCure. Electrochemical DAC company Phlair, formerly Carbon Atlantis, intends to partner with mineralization company Paebbl to store CO2 from one of their early DAC facilities as supplementary cementitious material. Other companies will likely follow Heirloom and Phlair’s footsteps in the near term while waiting for opportunities to tap into larger CO2 storage networks or secure access to dedicated wells.
Perform Small-Scale In Situ Mineralization
Class VI wells customarily involve injection into saline aquifers or depleted oil and gas wells where CO2 remains in a supercritical state before becoming a solid through mineral trapping over centuries to millennia. However, an emerging area of interest in the CO2 storage world concerns in situ mineralization, where CO2 is injected into ultramafic and mafic (categories of igneous) rock formations and forms solid carbonates. In this approach, CO2 is mixed with water before injection and reacts to form solid carbonates underground much more quickly than the traditional approach. Storage as a solid carbonate is highly desirable as it entails no reversal risk whatsoever, making promotion of this storage modality easier.
Three notable companies working in the in situ mineralization space are Carbfix, Cella, and 44.01, each of which has a variety of plans for testing, validating, and deploying their particular approaches. Climeworks has been working with Carbfix for several years to sequester just thousands of tons of CO2 produced from their Orca and Mammoth facilities in Iceland.
Cella is working with Kenyan DAC company Octavia Carbon on Project Hummingbird, which is expected to inject 1,000 tons per year of DAC CO2 underground for in situ mineralization. Due to the expected ease of storage access in Kenya, Climeworks and DAC startups Sirona Technologies and Yama are also exploring deployment options in the country with Great Carbon Valley.
The ability to synchronize in situ mineralization with mid-scale DAC capacities provides a compelling case for this approach, although there are still technical uncertainties, cost barriers, and permitting obstacles (at least in the U.S.) to overcome.
Utilize/Convert Captured CO2
EOR and mineralization are forms of carbon utilization or conversion, where captured CO2 is either used directly or converted in a way that yields a valuable product. There are many other CO2 utilization options given that most if not all carbon-containing chemicals can be made from CO2 with some reaction pathway (your economics may vary!) and that CO2 can be used directly in some applications.
Instead of finding a direct storage option, CO2 captured via DAC could be sold to utilizing entities offering goods and services ranging from jet fuel to methanol to greenhouse CO2 enrichment. This could provide a direct revenue source and capacities that could be better aligned with mid-scale DAC facilities.
For conversion of CO2 to relatively long-lived products such as graphite or durable plastics, captured and converted CO2 can represent both carbon removal and carbon conversion. Such pathways will struggle to sell premium carbon removal credits, however, as MRV protocols for these kinds of products do not really exist. One reason for this is the difficulty of tracking the fate of the captured carbon in carbon-utilizing products. Buyers want a reasonable assurance that CO2 they paid to be stored remains that way, but once something like a sheet of polycarbonate is sold into the market, there is no realistic means of individually tracking what ultimately happens to its carbon. While using DAC CO2 over petrochemicals in this case could still yield a climate benefit regardless of end-of-life treatment pending positive life cycle assessment results, it is different from high-confidence carbon removal.
DAC CO2 may also struggle to compete with cheaper natural sources of CO2, which applies primarily to EOR usage, and CO2 captured from high-concentration point sources such as ethanol and ammonia plants. Certain applications, such as pairing DAC and greenhouse CO2 enrichment, could be economically superior to separately capturing, conditioning, and transporting natural or point-source CO2, although the overall capacities and therefore climate impact of such approaches is limited.
Additionally, DAC technologies already pose a medium-to-high level of technical risk due to low technology readiness levels (TRLs), so stacking them with nascent carbon utilization processes could lead to an intolerable level of risk for investors. Developers investigating this strategy need to carefully consider and manage the technical and business risks associated with coupling their process with other nascent processes in a way that will best support future scale-up.
Despite the drawbacks and risks, there are multiple examples of companies trying this approach. Carbon Engineering investigated this option early on with their AIR TO FUELS process, Airhive intends to supply a Coca-Cola bottling facility with 1,000 tons of CO2 per year from a pilot facility, Skytree and DAC City are two notable DAC startups exploring the greenhouse CO2 market, RedoxNRG is investigating converting DAC CO2 to formic acid, and Porsche is working on converting DAC CO2 to fuels. Most other DAC companies have probably already considered sale into the industrial gas market or integration with carbon utilization companies as part of their roadmaps.
There are also several reactive carbon capture companies seeking to integrate DAC and CO2 conversion into one process, including Aerleum, Carbon Xtract, Homeostasis, and Susteon. These processes need to be designed in this way from the beginning but represent an additional category of DAC technologies that have found a potential solution to the near-term storage gap. Reactive carbon capture with DAC could also be considered part of the below category of companies that do not produce CO2 streams.
Avoid CO2 Production Altogether
Some companies are developing processes that look a lot like DAC but that do not produce pure streams of CO2 that have been isolated from the ambient air. Rather, the outputs from such systems are often carbonates intended either for direct disposition or utilization. Such systems are very similar to ex situ mineralization but do not involve intermediate CO2 production but rather produce carbonates directly.
Companies working on this approach include BluSky Carbon, Capture6, Carbon To Stone, Earth Repair, EDAC Labs, Equatic, Karbonetiq, Holy Grail, and Travertine. The technologies being developed by these companies are all at fairly low TRLs and are thus subject to change course on short notice.
By not producing pure CO2 and opting for solid carbonates, these processes avoid having to navigate the complexities of CO2 transportation and storage. However, by having unique processes distinct from most DAC approaches, they will face other challenges with aligning with existing MRV protocols, scaling to hundreds of megatons per year when considering the difficulties of moving copious amounts of solid waste, and 45Q tax credit qualification. In addition, like with reactive carbon capture, using this strategy generally entails designing it into the technology from the beginning. Given the customary need to reuse sorbents and solvents to reduce material costs, most DAC systems could not pivot to this approach.
Looking Forward
It is a challenging time to be a young DAC company. There is increasing pressure from all sides to build real plants, start storing CO2, and begin delivering real credits. Time is running out, both from a climate perspective and a runway perspective, making it hard to wait years for Class VI wells or their equivalents to be permitted and built.
There are several possible solutions as detailed here, but each one comes with trade-offs and may not be appropriate for any given technology or geography. Each DAC team needs to do their best to make it work, and it is incumbent on all other stakeholders in the industry ranging from investors to policymakers to help find reasonable and fair solutions to this problem.
If you have other ideas for how DAC developers might be able to solve the near-term storage gap, please share!
Nice review, but if DAC is storage limited you are missing one massive reservoir - the ocean. Obviously, it's not a good idea to add concentrated CO2 directly to seawater, which would be insecure and just further acidify the ocean. Rather, use "de-mineralization" to spontaneously convert CO2 to dissoved alkaline bicarbonate and carbonate ions to add to the marine pool of these that already compose the largest C reservoir in communication with the atmosphere (35,000 Gt C). Eg, CaCO3 + 1.7 CO2 + H2O --> Ca++ + 1.48HCO3- + 0.17CO3--.... This provides 70% more C storage per mol of alkaline rock than does mineralization (pure carbonate formation), plus helps counter ocean acidification. Coastally deploy DAC at sites near limestone and power with marine energy, no wells needed. Win, win, win?! Where's the DAC hub and $Bs for marine CO2 storage? greg@planetarytech.com
Thank you for writing this, very helpful!